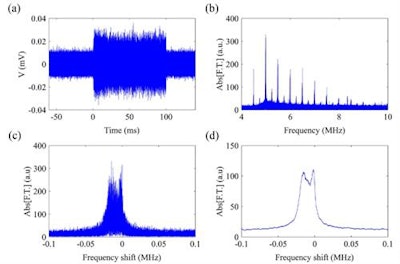
Several emerging therapies combine microbubbles with focused ultrasound, using them, for instance, to dissolve blood clots and open the blood-brain barrier for drug delivery. The therapeutic beams induce cavitation, the physical mechanism thought to be responsible for most of the biological effects, and exert a radiation force that produces microbubble motion. Techniques that monitor both are important in optimizing and controlling treatments.
In new work, U.K.-based researchers have estimated microbubble velocities during therapeutic ultrasound exposure. They did so by measuring the Doppler shift of ultrasound emitted by the microbubbles as they underwent cavitation. Uniquely, the measurements exploited high-order harmonics of the bubble emissions, called superharmonics, and are implementable in real-time with existing detectors already used to monitor cavitation.
"Images of [microbubble] spatial dynamics can be used by clinicians to understand where the treatment is occurring and how the treatment is evolving," said co-author James Choi, PhD, head of the Noninvasive Surgery & Biopsy Laboratory at Imperial College London. "This could lead to better patient responses from ultrasound therapies."
Measuring therapeutic beams
Ultrasound researchers have already developed techniques to measure several microbubble characteristics during ultrasound therapy, exploiting the acoustic emissions of the cavitating microbubbles. These include bubble location and the magnitude of cavitational activity, which can indicate, for example, the amount of drugs delivered. Bubble velocity is an additional, important parameter.
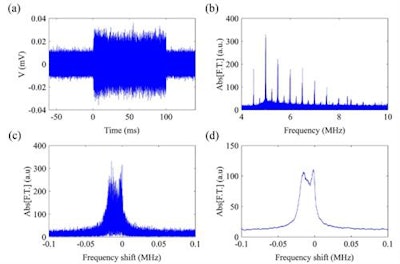
"The velocity at which [microbubbles] move determines the force induced by the collision [with the treatment target], and thus its success," said first author, PhD student Antonis Pouliopoulos. "This extra information of bubble velocity is extremely useful, since existing acoustic techniques are unable to estimate the stresses exerted during bubble therapy in real-time and in a passive manner."
Microbubble velocity has previously been measured with diagnostic ultrasound beams using pulse-echo techniques. However, these do not work with therapeutic beams. Of the order of milliseconds, therapeutic beam pulses are much longer than diagnostic pulses and overlap with microbubble emissions. Lower therapeutic frequencies also overlap with the fundamental resonant frequencies of the microbubbles.
Sidestepping these issues, Pouliopoulos and Choi set out to detect high harmonic components of the microbubble emissions and use their Doppler shifts to estimate bubble velocity. The superharmonics are those above the 10th harmonic, at much higher frequencies than therapeutic ultrasound beams. While microbubble superharmonics have been characterized before, no one has reported their Doppler shifts. The researchers discovered them serendipitously in unrelated microbubble research, when they observed a consistent drift in microbubble harmonics away from their expected frequency (Physics in Medicine and Biology, 2016, Vol. 61:16, pp. 6,154-6,171).
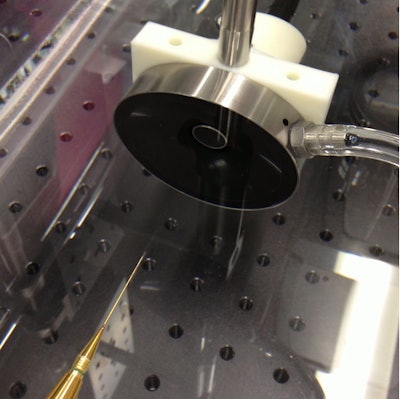
The Doppler shifts were measured using a microbubble-filled 4-mm diameter elastomer tube submerged in a water tank. A 0.5-MHz transducer driven by a waveform generator was used to sonicate the tube with 100-ms pulses. A smaller 7.5 MHz receive-only transducer, a so-called passive cavitation detector, was aligned co-axially with the transmit transducer.
A fast Fourier transform was used to convert the recorded signal into the frequency domain and quantify the microbubble superharmonics. A modified version of the Doppler equation was then applied to the measured frequency shifts to estimate the effective velocity of the microbubble population.
In exciting the microbubbles with lower frequency therapeutic pulses, the researchers generated stronger acoustic emissions than those obtained using a diagnostic beam. Doppler shifts also increase with harmonic frequency, and so both factors facilitated the velocity measurements.
Different order superharmonics returned consistent velocity values of up to 3 ms-1, varying according to the exact beam parameters used. The Doppler shifts were around 100 times those observed with a diagnostic beam. The higher shifts reflected the larger microbubble velocities, a result of the higher radiation force generated by the long beam pulses, and the larger shifts produced at higher frequencies.
In ongoing work, Choi and Pouliopoulos are extending the 1D measurement technique into 2D to generate acoustic maps of bubble velocity. They are also validating the maps with high-speed microscopy. Results so far are promising, Pouliopoulos told medicalphysicsweb.
© IOP Publishing Limited. Republished with permission from medicalphysicsweb, a community website covering fundamental research and emerging technologies in medical imaging and radiation therapy.